Proton Resonance Frequency Shift (PRFS) thermometry
This technique exploits the observation that the resonant frequency of protons in changes linearly with temperature across a clinically-relevant temperature range. The application of heat increases the kinetic energy and motion of water molecules causing an associated decrease in the average number of hydrogen bonds per unit volume. The protons experience greater electron shielding, thereby reducing the local magnetic field and decreasing the resonant frequency. It was first observed and reported by Hindman, and later applied in an MRI context by Ishihara and colleagues. The magnetic field seen by a proton can be described as:
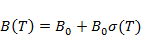
9
Where is the main field strength [T] and σ(T) is the chemical shift [ppm/°C ]. σ(T) can be further expanded as:

10
Where represents static variations in field strength, such as those arising from imperfections in the main field or differing tissue susceptibilities. σT(T) represents the temperature-dependent nuclear shielding coefficient for each proton. Note that because this analysis applies to all spins in the imaging volume, positional dependence has been disregarded. The phase of an image encodes the chemical shift:

11
Where γ is the gyromagnetic ratio for protons (42.58 MHz/T) and TE is the echo time. Gradient echo sequences must be used when thermometry information is desired. This is because the gradient echo does not refocus the phase accrued from the temperature dependent chemical shift. In contrast, a spin echo sequence refocuses the phase completely, cancelling the effect.
Expression (11) indicates that the final phase value is dependent on both the static chemical shift term and the temperature-dependent term. The static term can be eliminated straightforwardly by subtraction of a reference image in which no heating takes place:

12
This is known as the baseline subtraction method. is the complex reference image without heating, and Z is the complex image with heating. α is the temperature-dependent chemical shift proportionality for water (replacing the more general
(T) term). α is approximately 0.010 ppm/˚C . One of the primary strengths of the PRFS method is that this value is largely independent of tissue type , even though the variation in phase is very small and therefore easily obfuscated by other sources. The above formulation computes the relative change in temperature. For an absolute temperature a baseline temperature term is included:

13
Where represents a physical measurement of the initial temperature distribution across the imaging field of view (typically room temperature (22˚C) for phantom experiments, or 37˚C for in vivo measurements). Note that the phase value is limited to Δθ = 2π, and the appearance of TE in the denominator: A short TE increases the measureable range of ΔT but at the cost of decreased temperature resolution. Conversely, a long TE restricts the measureable range of ΔT but increases the temperature resolution. However a longer TE also reduces the image SNR as a result of T2 relaxation. The TE value that best balances these two effects and gives the maximum temperature SNR occurs when the echo time equals the T2* value of the tissue of interest. Unfortunately for a standard gradient echo sequence, typical T2* values (10 – 30ms) necessitate long TRs, which reduces the temporal resolution of the technique. This can be overcome to some extent through the use of a fast gradient echo sequence called echo planar imaging (EPI).
While the PRFS approach possesses many inherent advantages as an MR thermometry technique, it suffers from some fundamental limitations as well. Fat molecules cannot be imaged with this technique as they do not exhibit the same temperature dependent frequency shift aswater molecules. This can cause partial volume artefacts when voxels encompass a mixture of fat and water tissue. However this problem can be overcome with the use of fat suppression, though still does not solve the problem that no temperature measurements can be made in fatty tissue.
Because of the subtraction operation, registration artefacts arising from motion can be significant. Coupled with the use of EPI which is also motion-sensitive, steps must be taken to minimize motion. This can be accomplished through physical restraint of a subject (in the case of bulk patient motion), or with a variety of image-based techniques (see MRT Improvements). An important practical consideration is that phase data is typically wrapped, that is, restricted to values between [+π, – π]. Because of this the maximum possible Δθ is 2π. This puts a limit on the amount of temperature change that can be measured free of aliasing. For example at a field strength of B0 = 3T and a TE of 15ms, the maximum temperature change that can be measured is +/- 52.2˚C. The temperature elevation during HIFU exposures can often exceed this value, resulting in apparent cooling of tissue due to phase wrap. Therefore phase unwrapping techniques must be applied to acquired images prior to the subtraction step to remove this source of error.
PRFS data can be acquired simply and with much flexibility; its temperature measurements are accurate, and insensitive to tissue types. It is also currently the only non-invasive imaging method of thermometry accepted by the FDA for monitoring temperature during thermal therapy. Where complications exist, researchers have found effective methods for mitigation. Taken together, the PRFS approach is by far the most robust imaging method for monitoring thermal therapies.